Thermodynamic Properties of Substances: Understanding and Applying in Engineering Contexts
.webp)
Thermodynamics serves as the backbone of mechanical engineering, playing a pivotal role in shaping the principles and practices that govern the field. At its core, thermodynamics is the study of energy and its transformations, providing a fundamental framework for understanding the behavior of matter and energy in various engineering systems. The significance of thermodynamics in mechanical engineering cannot be overstated, as it forms the basis for the analysis, design, and optimization of a wide array of mechanical systems. If you find yourself in need of assistance with your Thermodynamics assignment, our experts are here to provide the support you need to navigate the intricacies of this fundamental discipline and excel in your studies.
In the realm of mechanical engineering, energy is a currency that fuels the operation of machines, from the smallest components to complex industrial systems. Thermodynamics provides the essential tools to comprehend how energy is transferred, converted, and utilized in these systems. This understanding is crucial for engineers to design efficient and sustainable solutions, considering factors such as performance, reliability, and environmental impact.
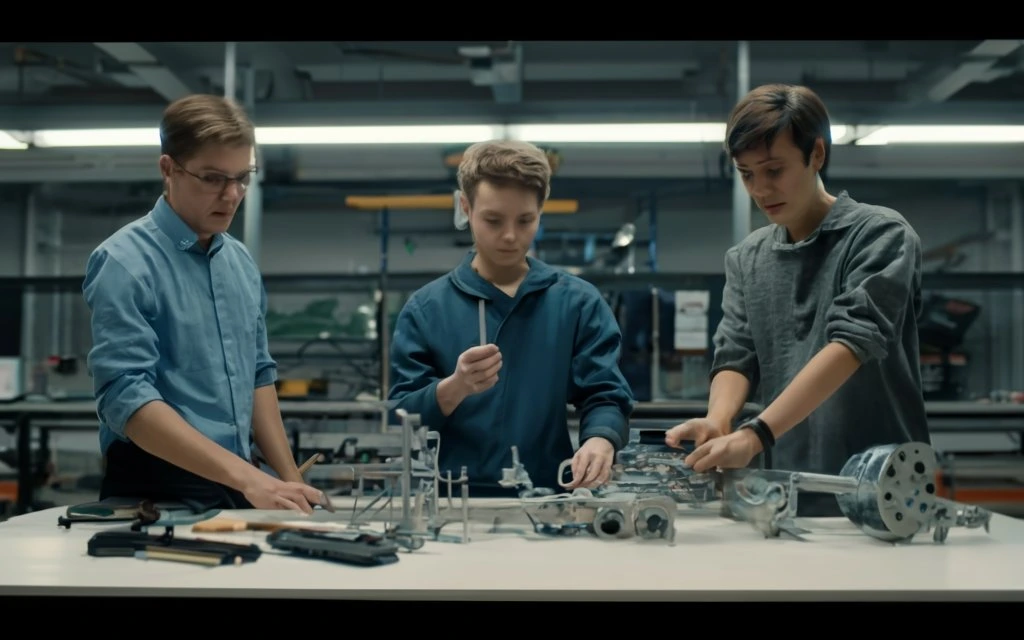
One of the primary contributions of thermodynamics to mechanical engineering lies in its ability to define and quantify thermodynamic properties of substances. Properties such as temperature, pressure, volume, and internal energy are fundamental building blocks for analyzing the behavior of materials and fluids within mechanical systems. Engineers leverage these properties to predict system responses, assess efficiency, and optimize performance in a variety of applications.
Understanding thermodynamic properties becomes particularly critical when dealing with heat engines, refrigeration systems, and power generation – key components in the mechanical engineering landscape. Thermodynamics allows engineers to evaluate the performance of these systems, ensuring that they operate within desired parameters and meet efficiency standards. Without a grasp of thermodynamic principles, the design and operation of these systems would be akin to navigating uncharted waters, leading to inefficiencies, increased energy consumption, and potential failures.
Moreover, thermodynamics provides a conceptual framework for comprehending the behavior of substances under different conditions. This knowledge is not only essential for theoretical analysis but also for practical applications. Engineers must predict how materials will behave in diverse environments, considering factors such as temperature variations, pressure changes, and heat transfer. Whether designing an aircraft engine, optimizing a manufacturing process, or developing a renewable energy system, a deep understanding of thermodynamics is indispensable for success.
In essence, thermodynamics is the cornerstone of mechanical engineering, providing the tools and principles necessary to unravel the complexities of energy systems. Its applications extend beyond theoretical analyses into the practical realm, influencing the design and operation of virtually every mechanical device. A solid grasp of thermodynamics empowers engineers to make informed decisions, ensuring that their creations not only function as intended but also contribute to the broader goals of efficiency, sustainability, and innovation in the field of mechanical engineering. As technology continues to advance, the role of thermodynamics remains ever relevant, guiding the next generation of engineers toward a future where mechanical systems are not only powerful but also intelligent and environmentally conscious.
Basics of Thermodynamics:
At its core, thermodynamics is the scientific study of energy and its transformations, providing a foundational framework for understanding the behavior of matter and energy within mechanical systems. In the realm of mechanical engineering, the significance of thermodynamics is paramount, serving as the guiding principles that govern the design, analysis, and optimization of a diverse range of engineering applications.
Fundamentally, energy is a central concept in thermodynamics. In the context of mechanical engineering, energy manifests in various forms, such as kinetic energy associated with motion, potential energy related to position, and internal energy inherent to the molecular structure of substances. The interconversion of these energy forms lies at the heart of mechanical systems, where engineers seek to harness and control energy to perform useful work.
Heat and work are two essential components in the thermodynamic landscape. Heat is the transfer of thermal energy between systems, typically driven by temperature differences. Work, on the other hand, is the application of force over a distance, resulting in the displacement of an object. In mechanical engineering, understanding the intricate relationship between heat and work is crucial for designing systems that efficiently convert energy from one form to another, whether in the operation of engines, refrigeration cycles, or power generation processes.
The laws of thermodynamics provide the overarching principles that govern energy transformations. The first law, often referred to as the Law of Conservation of Energy, asserts that energy cannot be created nor destroyed; it can only change forms. In the context of mechanical engineering, this law is foundational for ensuring that the total energy input to a system is equal to the useful work output and the energy lost as heat.
The second law introduces the concept of entropy, a measure of the disorder or randomness in a system. It states that in any energy transformation, the overall entropy of an isolated system tends to increase over time. This law has profound implications for the efficiency of mechanical systems, influencing the design choices engineers make to minimize energy losses and maximize useful work output.
In summary, the basics of thermodynamics are indispensable in mechanical engineering, providing the theoretical underpinning for the design and analysis of energy systems. Through the understanding of energy, heat, work, and the laws of thermodynamics, engineers gain the tools necessary to create efficient, sustainable, and innovative solutions, shaping the future of mechanical engineering practice.
Thermodynamic Properties of Substances:
In the expansive realm of thermodynamics, key properties serve as fundamental parameters for characterizing and analyzing substances within mechanical systems. Temperature, pressure, and volume stand out as cornerstones, wielding immense importance in understanding and predicting the behavior of materials and fluids.
Temperature, a measure of the average kinetic energy of particles in a substance, plays a pivotal role in determining the direction of heat transfer and influencing the overall energy state of a system. In mechanical engineering, precise control and manipulation of temperature are critical, whether in the design of combustion engines, refrigeration cycles, or industrial processes.
Pressure, the force exerted per unit area, is equally crucial. It governs fluid flow, combustion processes, and the structural integrity of mechanical components. Understanding and managing pressure variations are essential for ensuring the safety and efficiency of systems ranging from hydraulic machinery to gas turbines.
Volume, the amount of space occupied by a substance, intertwines with temperature and pressure to define the state of a system. Changes in volume impact the thermodynamic processes within mechanical systems, guiding the design of devices such as compressors, pumps, and heat exchangers.
Thermodynamic properties can further be classified into intensive and extensive properties, each carrying distinct implications. Intensive properties, such as temperature and pressure, are independent of the size or quantity of the system. They offer insights into the internal state of the material, facilitating comparisons and analyses across different scales. Extensive properties, like volume and mass, depend on the size or quantity of the system. They describe the overall magnitude of a property and are crucial for assessing the total energy content or entropy of a system.
Integral to the understanding of thermodynamics is the concept of state functions. These are properties that depend only on the current state of the system, not on the path it took to reach that state. Temperature, pressure, and internal energy are examples of state functions. This concept is instrumental in simplifying the analysis of complex thermodynamic processes, allowing engineers to focus on initial and final states rather than intricate details of the intermediate steps.
In conclusion, the comprehension of key thermodynamic properties, including temperature, pressure, and volume, is indispensable for mechanical engineers. Intensive and extensive properties offer a nuanced perspective, enabling precise system characterization, while the concept of state functions streamlines the analysis of thermodynamic processes. These properties collectively empower engineers to design, optimize, and troubleshoot a myriad of mechanical systems, laying the foundation for innovation and efficiency in the field of mechanical engineering.
Types of Thermodynamic Processes:
Thermodynamic processes form the conceptual framework that underpins the understanding of how energy is transformed and exchanged within physical systems, playing a central role in disciplines such as mechanical engineering, physics, and chemistry. These processes describe the changes that a system undergoes concerning its thermodynamic state variables, notably temperature, pressure, and volume. At the heart of these processes lies the first law of thermodynamics, the principle of energy conservation, which asserts that energy cannot be created or destroyed but only transferred or converted from one form to another. This foundational law serves as the cornerstone for comprehending the intricacies of various thermodynamic processes.
An essential thermodynamic process is the isothermal process, where the temperature remains constant during the transformation. In practical terms, this occurs when a system is in thermal equilibrium with its surroundings, allowing heat exchange without a change in temperature. Adiabatic processes, on the other hand, occur without the transfer of heat, a concept central to understanding rapid, non-equilibrium transformations. Isobaric processes maintain a constant pressure, while isochoric processes occur at constant volume. These processes, collectively, provide a comprehensive toolkit for engineers and scientists to analyze and design systems with precision.
Moreover, thermodynamic processes are categorized as reversible or irreversible, with profound implications for system efficiency. A reversible process is one that can be reversed without leaving any impact on the surroundings or the system itself. In contrast, irreversible processes, once completed, result in changes that cannot be undone without affecting the system or its surroundings. The irreversibility of processes introduces the concept of entropy, a measure of the system's disorder or randomness, emphasizing the directionality of natural processes and the tendency of systems toward greater disorder.
In the world of mechanical engineering, thermodynamic processes are omnipresent. From the compression and expansion strokes in internal combustion engines to the intricate cycles within refrigeration systems, engineers rely on a deep understanding of these processes to design, optimize, and troubleshoot mechanical systems. The application of thermodynamic principles extends beyond engineering into fields such as environmental science, astrophysics, and material science, highlighting the universality and versatility of these foundational concepts. In essence, thermodynamic processes provide the language and tools necessary for scientists and engineers to navigate the complexities of energy transformations, offering insights that shape technological advancements and pave the way for sustainable and innovative solutions in the modern world.
Isothermal Process:
An isothermal process, a fundamental concept in thermodynamics, characterizes a scenario in which the temperature of a system remains constant as it undergoes a change. This implies that the internal energy of the system remains constant throughout the process. The significance of isothermal processes lies in their practical applications and theoretical implications, particularly in understanding the behavior of gases and the principles governing heat exchange.
In an isothermal process, a notable example is the expansion or compression of a gas within a heat reservoir. A heat reservoir is an infinite source or sink of heat that can maintain a constant temperature, allowing the system to exchange heat with its surroundings without altering its own temperature. When a gas expands, for instance, it does work on its surroundings, and to maintain an unchanging temperature, heat is absorbed from the reservoir. Conversely, during compression, work is done on the gas, and excess heat is released into the reservoir, keeping the temperature constant.
A classic illustration of an isothermal process is the operation of a Carnot heat engine, often used as an idealized benchmark for maximum thermodynamic efficiency. In the isothermal expansion stage of the Carnot cycle, the working substance (often a gas) absorbs heat from the high-temperature reservoir while expanding, maintaining a constant temperature. This adherence to isothermality allows for a more efficient conversion of heat into work, highlighting the practical relevance of isothermal processes in optimizing energy systems.
The isothermal process stands in contrast to adiabatic processes, where no heat exchange occurs with the surroundings. While isothermal processes are idealized and often approached in real-world situations, their study provides crucial insights into the thermodynamic behavior of systems and serves as a foundational concept in the analysis and design of various engineering applications. Engineers leverage the understanding of isothermal processes to enhance the efficiency of heat engines, refrigeration cycles, and other systems where temperature control is paramount, showcasing the practical importance of this thermodynamic principle in shaping the advancements of modern technology.
Adiabatic Process:
An adiabatic process, a fundamental concept in thermodynamics, characterizes a transformation in a system that occurs without the transfer of heat between the system and its surroundings. In essence, during an adiabatic process, the system is thermally isolated, preventing any exchange of heat with its environment. This isolation implies that the internal energy of the system changes solely due to work done on or by the system. The practical significance of adiabatic processes is particularly evident in scenarios where rapid changes in pressure and temperature occur.
A compelling example of an adiabatic process can be found in the operation of a pneumatic tool, such as an air compressor or a jackhammer. In these applications, air undergoes rapid compression or expansion within the tool, and the process happens quickly enough to inhibit significant heat exchange with the surroundings. When air is compressed adiabatically, it experiences an increase in temperature due to the compression work done on it. Similarly, during adiabatic expansion, the air cools as it does work on the surroundings. The speed at which these processes occur is crucial; if the compression or expansion were slow, heat exchange with the surroundings would be more pronounced, and the process would deviate from adiabatic conditions.
The adiabatic process is a key component in the operation of internal combustion engines, where air-fuel mixtures undergo rapid compression and expansion strokes. In these engines, the prevention of heat transfer during these strokes is essential for maximizing efficiency and power output. Additionally, adiabatic processes are integral to the functioning of gas turbines and various industrial applications where precise control over temperature changes is paramount.
Understanding adiabatic processes is not only fundamental for engineers designing efficient machinery but also plays a critical role in astrophysics and atmospheric science. Adiabatic cooling and heating processes contribute to phenomena such as cloud formation and the adiabatic lapse rate in Earth's atmosphere. In summary, the concept of adiabatic processes is a cornerstone in thermodynamics, offering insights into the behavior of systems undergoing rapid and heat-isolated transformations, with wide-ranging applications across engineering and the natural sciences.
Isobaric Process:
An isobaric process, a fundamental concept in thermodynamics, delineates a scenario in which the pressure of a system remains constant throughout a change, while other thermodynamic properties may vary. The term "isobaric" is derived from the Greek words iso, meaning equal, and baros, meaning weight or pressure, reflecting the characteristic constancy of pressure during this type of transformation. This process is particularly insightful for understanding the behavior of substances as they respond to external factors, offering valuable insights into real-world applications where pressure control is crucial.
A compelling example of an isobaric process can be observed in the heating of water in an open container. As heat is applied to the water, the pressure within the container remains constant, typically at atmospheric pressure. This constancy in pressure allows the water to undergo a phase change, transitioning from a liquid to vapor as it reaches its boiling point. Throughout this transformation, the pressure remains unchanged, emphasizing the isobaric nature of the process. In practical terms, this phenomenon is familiar in everyday activities such as cooking, where water boils at a consistent pressure, and the temperature remains steady until all the liquid has converted to vapor.
The isobaric process stands in contrast to isochoric (constant volume) and isothermal (constant temperature) processes, providing a distinct perspective on how systems respond to external influences. In engineering applications, understanding isobaric processes is essential for designing systems where pressure needs to be maintained within specific bounds. For example, in chemical engineering, reactors often operate under isobaric conditions to ensure consistency in pressure during chemical reactions. Additionally, isobaric processes play a role in air-conditioning systems, where maintaining a constant pressure ensures effective and controlled heat exchange.
The isobaric process also finds relevance in atmospheric science. As air rises or descends in the atmosphere, it may undergo isobaric expansion or compression, influencing temperature and contributing to the formation of weather patterns. In essence, the isobaric process provides a valuable framework for engineers and scientists to comprehend and manipulate systems where pressure plays a critical role, offering insights into diverse applications across various fields.
Isochoric Process:
An isochoric process, often referred to as an isovolumetric process, is a fundamental concept in thermodynamics that characterizes a system undergoing a transformation at constant volume. The term is derived from the Greek words "iso," meaning equal, and "Chora," meaning space, highlighting the characteristic of an unchanging volume during this process. Isochoric processes are particularly insightful in understanding the influence of energy interactions within a confined space and find relevance in various applications, including combustion and certain experimental conditions.
A noteworthy example of an isochoric process is observed during combustion in a sealed container. In this scenario, the volume of the container remains constant as a result of the confinement, preventing any expansion or contraction. When a combustible material undergoes a chemical reaction, such as the combustion of a fuel, the release of energy in the form of heat leads to an increase in temperature and pressure within the container. However, since the volume is held constant, the energy released during the reaction contributes solely to the thermal aspects of the system. This makes isochoric processes particularly relevant in understanding the specific heat capacities of substances and the thermal dynamics associated with chemical reactions.
The isochoric process stands in contrast to isobaric (constant pressure) and isothermal (constant temperature) processes, providing a unique perspective on how energy is exchanged within a system. In experimental setups, scientists may deliberately employ isochoric conditions to study the heat capacities of substances without the complicating factors associated with changes in volume. This controlled environment allows for a more precise analysis of the energy interactions and thermal characteristics of materials.
Understanding isochoric processes is pivotal in the design and analysis of various systems, including engines and reactors, where controlled volumes are integral. In internal combustion engines, for instance, the combustion of fuel-air mixtures occurs at constant volume during specific phases of the engine cycle. Additionally, isochoric processes find applications in experimental setups where researchers seek to isolate and investigate the thermal properties of substances. In essence, the isochoric process provides valuable insights into the intricacies of energy interactions in systems where volume remains constant, contributing to advancements in both fundamental thermodynamics and practical engineering applications.
Applications in Mechanical Engineering:
The comprehension of thermodynamic properties is of paramount importance in the realm of mechanical engineering, as it lays the foundation for designing and optimizing a myriad of systems. One significant application is in the design and operation of heat exchangers, where the understanding of properties like temperature, pressure, and heat transfer coefficients is critical. Heat exchangers are ubiquitous in engineering systems, ranging from HVAC systems to industrial processes. Engineers leverage thermodynamic principles to ensure efficient heat transfer between fluids, optimizing the performance of these devices by selecting appropriate materials, configurations, and operating conditions.
In the domain of engines, be it internal combustion engines or gas turbines, the role of thermodynamic properties is indispensable. The efficiency and power output of engines directly correlate with the understanding and manipulation of properties such as temperature and pressure during the combustion and expansion processes. Engineers strive to maximize work output while minimizing energy losses, and a deep understanding of thermodynamic properties enables the precise control needed for optimal engine performance. From the compression stroke in internal combustion engines to the complex cycles within gas turbines, thermodynamics guides engineers in the pursuit of efficient and powerful mechanical systems.
Refrigeration systems, central to various industries and daily life, provide another compelling application of thermodynamic principles in mechanical engineering. The behavior of refrigerants, their phase changes, and the transfer of heat in these systems are governed by thermodynamic properties. Engineers design refrigeration cycles with meticulous attention to these properties to achieve desired cooling effects. Whether in household refrigerators, air conditioning units, or industrial-scale refrigeration, the mastery of thermodynamics is fundamental to achieving energy-efficient and environmentally friendly cooling solutions.
Furthermore, thermodynamic properties play a pivotal role in the design of power plants, where the conversion of heat to electricity involves intricate processes. From steam cycles in traditional power plants to more advanced technologies like combined cycle plants, understanding properties such as entropy, enthalpy, and specific heat is essential for optimizing energy conversion and minimizing waste. Engineers employ thermodynamic principles to enhance the efficiency of power generation systems, contributing to the sustainable and economical production of electricity.
In conclusion, the application of thermodynamic properties in mechanical engineering is vast and diverse. Whether in the design of heat exchangers, engines, refrigeration systems, or power plants, a profound understanding of thermodynamics empowers engineers to create systems that are not only efficient but also innovative and environmentally conscious. Thermodynamics serves as the guiding framework that shapes the development of mechanical systems, fostering advancements that contribute to a more sustainable and technologically advanced future.
Tools and Software for Thermodynamic Analysis:
In the realm of thermodynamics, a plethora of tools and software have become indispensable for engineers and students seeking to analyze and model complex systems. One prominent tool is MATLAB, a high-level programming language that offers powerful capabilities for numerical analysis, data visualization, and mathematical modeling. MATLAB's extensive library of built-in functions, along with toolboxes dedicated to thermodynamics and fluid mechanics, enables users to perform simulations, solve equations, and conduct parametric studies to gain valuable insights into thermodynamic properties. Students and engineers can leverage MATLAB for tasks such as analyzing heat exchangers, simulating thermodynamic cycles, and optimizing system performance.
Another widely utilized software in the field is Engineering Equation Solver (EES). EES is specifically designed for solving and analyzing thermodynamic problems, offering a user-friendly interface that simplifies complex calculations. It provides a vast library of built-in thermophysical properties and equations, allowing users to easily set up and solve problems related to heat transfer, fluid flow, and energy conversion. Engineers and students can employ EES to explore different scenarios, conduct sensitivity analyses, and validate their design calculations, making it an invaluable tool for both learning and practical applications.
For those involved in more specialized thermodynamic analysis, Computational Fluid Dynamics (CFD) software such as ANSYS Fluent or COMSOL Multiphysics is highly advantageous. These tools enable engineers to simulate and visualize fluid flow, heat transfer, and other thermodynamic processes within intricate geometries. With these software packages, users can assess the performance of heat exchangers, combustion systems, and various fluidic devices under different operating conditions. The visualization capabilities of CFD software allow engineers to gain insights into complex fluid dynamics, aiding in the optimization of system designs.
Additionally, thermodynamic software like REFPROP provides a comprehensive database of thermophysical properties for a wide range of fluids. This software is particularly valuable for engineers dealing with refrigeration systems, where precise knowledge of properties like enthalpy, entropy, and specific heat is crucial for system design and analysis.
To leverage these tools effectively, students and engineers can benefit from online courses, tutorials, and documentation provided by the software developers. Many universities and organizations offer training programs or workshops to enhance proficiency in these tools. Furthermore, engaging in practical projects and real-world applications can solidify the understanding of thermodynamic principles and the use of these tools.
In conclusion, the availability of advanced tools and software has revolutionized the way engineers and students analyze thermodynamic properties. MATLAB, EES, CFD software, and thermophysical property databases offer diverse capabilities that cater to different aspects of thermodynamic analysis. By acquiring proficiency in these tools through education and hands-on experience, students and engineers can enhance their problem-solving skills, conduct sophisticated analyses, and contribute to the development of innovative and efficient thermodynamic systems.
Conclusion:
In conclusion, the blog post delves into the intricacies of thermodynamic properties, highlighting their fundamental role in the field of mechanical engineering. The exploration begins with a comprehensive overview of thermodynamics, elucidating its significance as the science of energy and its transformations. The basics of thermodynamics, encompassing concepts such as energy, heat, and work, are introduced, setting the stage for a deeper understanding of the subject. The subsequent sections shed light on key thermodynamic processes, including isothermal, adiabatic, isobaric, and isochoric processes, each accompanied by real-world examples that illustrate their applications and relevance in various engineering contexts.
The discussion extends to the importance of thermodynamic properties, focusing on temperature, pressure, and volume, and their role in the design and operation of mechanical systems. The isothermal process, where temperature remains constant, finds application in refrigeration systems, while adiabatic processes, occurring without heat transfer, are crucial in the efficiency of engines and industrial processes. Isobaric processes, maintaining constant pressure, are vital in scenarios such as heating water, and isochoric processes, where volume remains constant, are instrumental in understanding combustion dynamics in engines.
The blog post also touches upon the tools and software available for thermodynamic analysis, showcasing how MATLAB, Engineering Equation Solver (EES), and Computational Fluid Dynamics (CFD) software empower engineers and students to simulate, analyze, and optimize thermodynamic systems. These tools provide a bridge between theoretical knowledge and practical applications, enabling the exploration of complex scenarios and the optimization of system performance.
In the final analysis, the overarching theme emphasizes the critical role of a solid understanding of thermodynamic properties for success in mechanical engineering assignments and projects. The mastery of thermodynamics is portrayed as essential for designing efficient systems, analyzing complex processes, and addressing real-world challenges. From the design of heat exchangers and engines to the operation of refrigeration systems and power plants, thermodynamic principles guide engineers in creating innovative and sustainable solutions. The depth of knowledge in thermodynamics, coupled with proficiency in relevant tools and software, equips students and engineers with the expertise needed to contribute meaningfully to the advancement of mechanical engineering, where efficiency, precision, and innovation are paramount. In essence, a solid understanding of thermodynamic properties is the cornerstone upon which successful mechanical engineering endeavors are built.